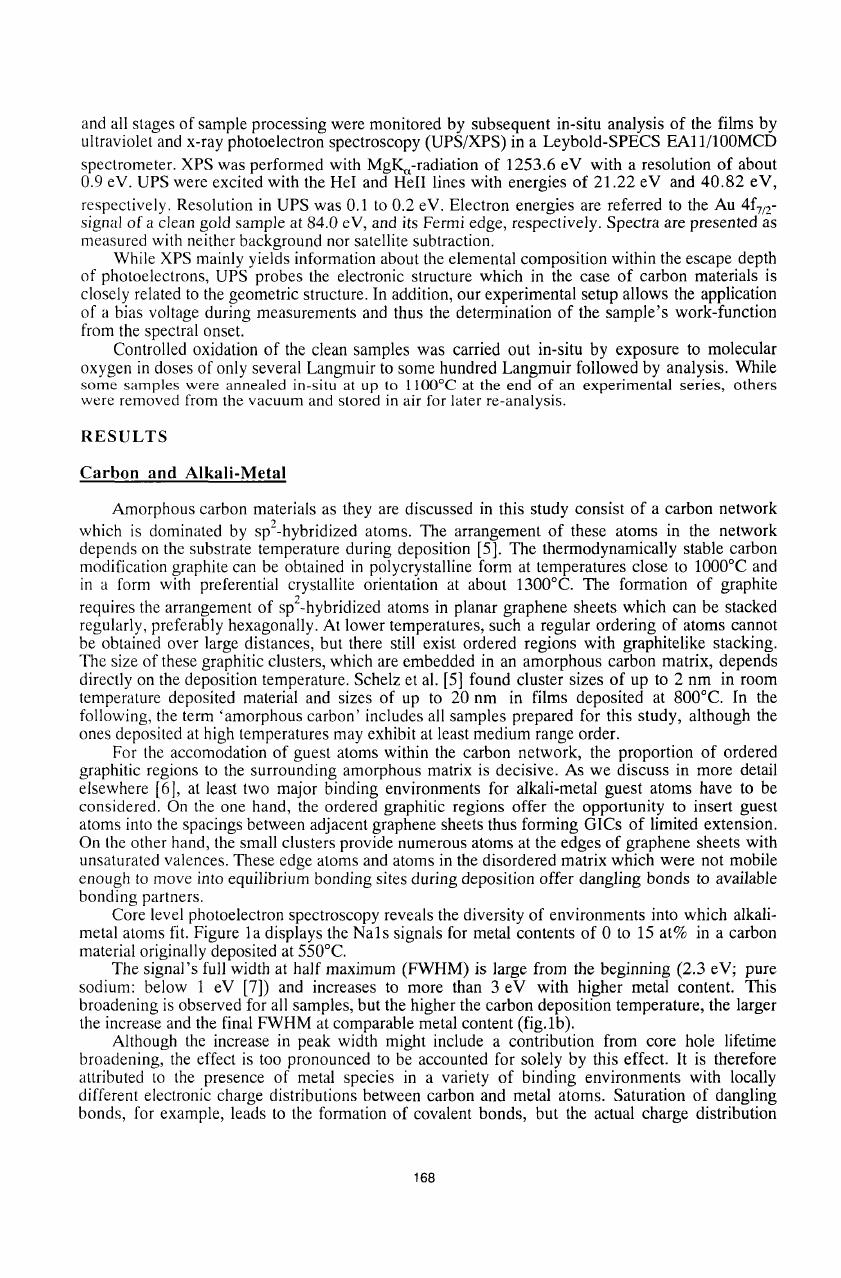
and
all
stages
of
sample
processing
were
monitored
by
subsequent
in-situ analysis
of
the
films
by
ultraviolet
and
x-ray
photoelectron
spectroscopy (UPS/XPS)
in
a
Leybold-SPECS
EA11/100MCD
spectrometer.
XPS
was
performed with
MgK
0
-radiation
of
1253.6
eV
with
a
resolution
of
about
0.9
eV.
UPS
were
excited
with
the
Hel
and
Hell
lines
with
energies
of 21.22
eV
and
40.82
eV,
respectively. Resolution
in
UPS
was
0.1
to
0.2
eV.
Electron
energies
are
referred
to
the
Au
4f
7
/
2
-
signal
of
a
clean
gold
sample
at
84.0
eV,
and
its
Fermi
edge,
respectively.
Spectra
are
presented
as
measured with neither
background
nor
satellite
subtraction.
While
XPS
mainly yields
information
about
the
elemental
composition
within
the
escape
depth
of
photoelectrons,
UPS
probes
the
electronic
structure
which
in
the
case
of
carbon materials
is
closely
related
to
the
geometric
structure.
In
addition,
our
experimental
setup
allows
the
application
of
a
bias voltage
during measurements
and
thus
the
determination
of
the
sample's
work-function
from
the
spectral
onset.
Controlled oxidation
of
the
clean
samples
was
carried
out in-situ by
exposure
to
molecular
oxygen
in
doses
of
only
several
Langmuir
to
some hundred
Langmuir
followed
by
analysis.
While
some
samples
were
annealed
in-situ
at
up
to
1
100°C
at
the
end
of
an
experimental
series,
others
were
removed
from
the
vacuum
and
stored
in
air for
later
re-analysis.
RESULTS
Carbon
and
Alkali-Metal
Amorphous
carbon
materials
as
they
are
discussed
in
this
study
consist
of
a
carbon
network
which
is
dominated
by
spZ-hybridized
atoms.
The
arrangement
of
these
atoms
in
the
network
depends
on the
substrate
temperature
during
deposition
[5].
The
thermodynamically
stable
carbon
modification
graphite
can
be
obtained
in
polycrystalline
form
at
temperatures
close
to
1000°C
and
in
a
form
with
preferential
crystallite
orientation
at
about
1300'C.
The
formation
of
graphite
requires
the
arrangement
of
sp
2
-hybridized
atoms
in
planar
graphene
sheets
which
can
be
stacked
regularly,
preferably
hexagonally.
At lower
temperatures,
such
a
regular
ordering
of
atoms
cannot
be
obtained over
large
distances,
but
there
still
exist
ordered
regions
with
graphitelike
stacking.
The
size
of
these
graphitic clusters,
which
are
embedded
in
an
amorphous
carbon
matrix,
depends
directly
on the
deposition
temperature.
Schelz
et
al.
[5]
found cluster
sizes
of
up
to
2
nm
in
room
temperature
deposited
material
and
sizes
of
up
to
20 nm
in
films
deposited
at
800'C.
In
the
following,
the
term
'amorphous
carbon'
includes
all
samples
prepared for
this
study,
although
the
ones
deposited
at
high
temperatures
may
exhibit
at
least
medium
range
order.
For
the
accomodation
of
guest
atoms within
the
carbon
network,
the
proportion
of
ordered
graphitic
regions
to
the
surrounding
amorphous
matrix
is
decisive.
As
we
discuss
in
more
detail
elsewhere
[6],
at
least
two
major
binding
environments
for
alkali-metal
guest
atoms
have
to
be
considered.
On
the
one hand,
the
ordered graphitic
regions
offer
the
opportunity
to
insert guest
atoms
into
the
spacings between adjacent
graphene
sheets thus
forming
GICs
of
limited
extension.
On
the
other
hand,
the
small
clusters
provide numerous
atoms
at
the
edges
of
graphene sheets
with
unsaturated
valences. These
edge
atoms
and
atoms
in
the
disordered
matrix which were
not
mobile
enough
to
move
into
equilibrium
bonding
sites
during deposition offer
dangling
bonds
to
available
bonding
partners.
Core
level
photoelectron spectroscopy
reveals
the
diversity
of
environments
into
which
alkali-
metal
atoms
fit.
Figure
Ia
displays
the
Nals
signals
for
metal
contents
of
0
to
15
at%
in
a
carbon
material
originally
deposited
at
550'C.
The
signal's
full
width
at
half
maximum
(FWHM)
is
large
from
the
beginning
(2.3
eV;
pure
sodium:
below
1
eV
[7])
and
increases
to
more
than
3
eV
with
higher
metal
content.
This
broadening
is
observed
for
all
samples,
but
the
higher
the
carbon
deposition
temperature,
the
larger
the
increase
and
the
final
FWHM
at
comparable
metal
content
(fig.
b).
Although
the
increase
in
peak
width
might
include
a
contribution
from
core hole
lifetime
broadening,
the
effect
is
too
pronounced
to
be
accounted
for
solely
by
this
effect.
It
is
therefore
attributed
to
the
presence
of
metal
species
in
a
variety
of
binding
environments
with
locally
different
electronic
charge
distributions
between carbon
and
metal
atoms.
Saturation
of
dangling
bonds,
for
example,
leads
to
the
formation
of
covalent
bonds,
but
the
actual
charge
distribution
168