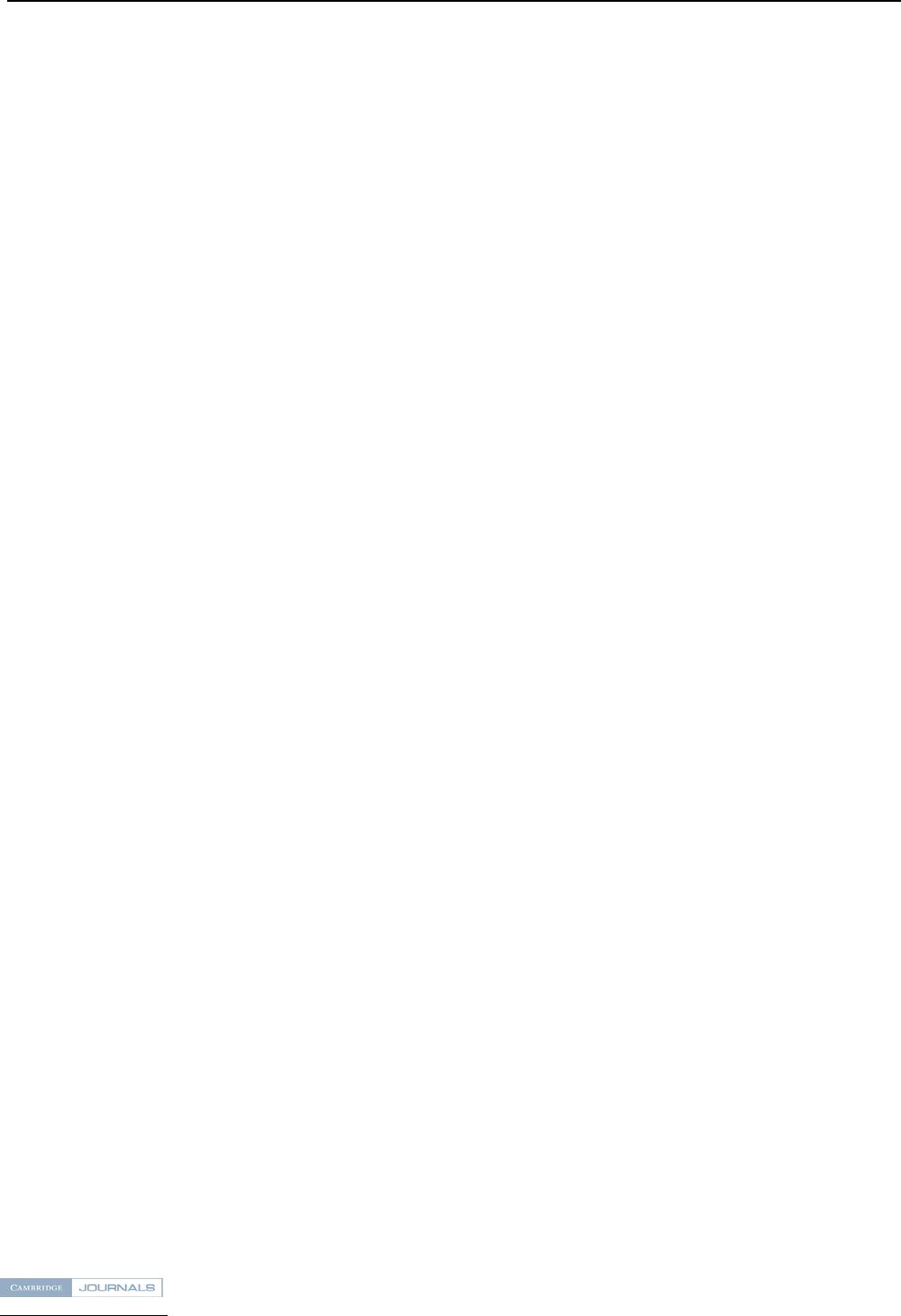
http://journals.cambridge.org Downloaded: 18 Mar 2015 IP address: 128.255.6.125
K.L. Saenger
et al.:
Noble metal silicide formation in metalySi structures
fulness of Ti is expected to be limited by its propensity
for diffusing to the Ir top surface in IryTiySiO
2
structures
where the Ti may oxidize. Such effects were observed
in the present work and previously for Ti with Pt.
16,17
The differences in the relative amounts of noble
metal silicide and unreacted noble metal left after oxygen
annealing in the RhySi, PtySi, and IrySi samples reflect
differences in the competition between (i) silicidation
and (ii) oxygen diffusion to and pileup at the reaction
front between the silicided and unreacted noble metal.
Silicide formation is fastest with RhySi and slowest with
IrySi. Both Rh and Pt are expected to be permeable
to oxygen; Rh is reported
18
to absorb oxygen when
melted, and Pt is reported
18
to absorb oxygen when
heated in air. Surface IrO
2
formation rather than oxygen
absorption is reported for Ir.
18
Our data thus suggest
that the Rh silicides before enough oxygen is absorbed to
have an effect. Oxygen has more of an effect on the more
slowly siliciding PtySi, perhaps because platinum silicide
formation is still in progress at temperatures high enough
for Pt to start acting as a sponge for oxygen. Silicidation
in IrySi is even slower, but Ir is also less permeable to
oxygen. However, the relative abundance of unreacted Ir
versus the iridium silicide clearly indicates that oxygen
is reaching the reaction front in sufficient amounts to
stop the silicidation reaction. It can thus be concluded
that the surface IrO
2
is either permeable to oxygen, or
does not become thick enough to interfere with oxygen
diffusion until after the silicidation reaction has stopped.
V. CONCLUSIONS
This paper compared noble metal silicide formation
in structures of PtySi, RhySi, IrySi, and IryTiySi annealed
in oxygen or nitrogen at 640
±
C for 6 min, the noble
metal having an initial thickness of about 100 nm. While
complete silicidation was observed in the RhySi, PtySi,
and IrySi samples after nitrogen annealing, some Pt
and most of the Ir remained after equivalent anneals
in oxygen. In contrast to the low resistivity silicides
formed from RhySi (orthorhombic RhSi, with a resistiv-
ity ,140 mV –cm) and PtySi (orthorhombic PtSi, with
a resistivity ,45 mV –cm), the iridium silicide formed
from IrySi is the semi-conducting IrSi
1.75
with a very
high resistivity (,1–5 V–cm).
The formation of the IrSi
1.75
silicide can be delayed,
although not prevented, with the use of a 5 nm Ti
adhesion layer. In this case, unreacted Ir remained after
both nitrogen and oxygen anneals, with XRD showing no
silicide formation after oxygen annealing and formation
of the conductive (,500 mV –cm) orthorhombic IrSi
after nitrogen annealing.
ACKNOWLEDGMENTS
The authors thank Roy Carruthers for the film dep-
ositions, G. Coleman for the RBS measurements, and
F. d’Heurle for a helpful discussion.
REFERENCES
1. A. Grill and M.J. Brady, Integrated Ferroelectrics 9, 299 (1995).
2. C.E. Allevato and C. B. Vining, J. Alloys Compounds 200,99
(1993).
3. J. Schumann, D. Elefant, C. Glandun, A. Heinrich, W. Pitschke,
H. Lange, W. Henrion, and R. Grotzschel, Phys. Status Solidi A
145, 429 (1994).
4. S. Petersson, J. A. Reimer, M. H. Brodsky, D. R. Campbell,
F. d’Heurle, B. Karlsson, and P. A. Tove, J. Appl. Phys. 53, 3342
(1982).
5. C. Canali, C. Catellani, M. Prudenziati, W. H. Wadlin, and C. A.
Evans, Jr., Appl. Phys. Lett. 31, 43 (1977).
6. M. Wittmer, J. Appl. Phys. 54, 5081 (1983).
7. C. Harder, L. Hammer, and K. Muller, Phys. Status Solidi A 146,
385 (1994).
8. S.R. Das, K. Sheergar, D-X. Xu, and A. Naem, Thin Solid Films
253, 467 (1994).
9. T. Rodriguez, H. Wolters, A. Almendra, J. Sanz-Maudes, M. F.
DaSilva, and J.C. Soares, in Infrared Detectors—Materials, Proc-
essing, and Devices, edited by A. Appelbaum and L.R. Dawson
(Mater. Res. Soc. Symp. Proc. 299, Pittsburgh, PA, 1994), p. 313.
10. S. Petersson, J. Baglin, W. Hammer, F. d’Heurle, T. S. Kuan,
I. Ohdomari, J. de Sousa Pires, and P. Tove, J. Appl. Phys. 50,
3357 (1979).
11. M. Wittmer, P. Oelhafen, and K. N. Tu, Phys. Rev. B 35, 9073
(1987).
12. S. Petersson, R. Anderson, J. Baglin, J. Dempsey, W. Hammer,
F. d’Heurle, and S. LaPlaca, J. Appl. Phys. 51, 373 (1980).
13. M. Fernandez, T. Rodriguez, A. Almendra, J. Jimenez-Leube,
and H. Wolters, in Infrared Detectors—Materials, Processing,
and Devices, edited by A. Appelbaum and L.R. Dawson (Mater.
Res. Soc. Symp. Proc. 299, Pittsburgh, PA, 1994), p. 325.
14. R.A. Roy, L. A. Clevenger, C. Cabral, Jr., K. L. Saenger,
S. Brauer, J. Jordan-Sweet, J. Bucchignano, G.B. Stephenson,
G. Morales, and K. F. Ludwig, Jr., Appl. Phys. Lett. 66, 1732
(1995).
15. L. Krusin-Elbaum, M. Wittmer, and D. S. Yee, Appl. Phys. Lett.
50, 1879 (1987).
16. R. Bruchhaus, D. Pitzer, O. Eibl, U. Scheithauer, and W. H
¨
osler,
in Ferroelectric Thin Films II, edited by A. I. Kingon, E.R. Myers,
and B. Tuttle (Mater. Res. Soc. Symp. Proc. 243, Pittsburgh, PA,
1992), p. 123.
17. R.E. Jones, Jr., P. D. Maniar, J. L. Dupuie, J. Kim, R. Moazzami,
J. Witowski, M.L. Kottke, N. C. Saha, and R. B. Gregory, in
Ferroelectric Thin Films IV, edited by B. A. Tuttle, S. B. Desu,
R. Ramesh, and T. Shiosaki (Mater. Res. Soc. Symp. Proc. 361,
Pittsburgh, PA, 1995), p. 223.
18. The Merck Index, 10th ed., edited by M. Windholz (Merck, Inc.,
1983).
468 J. Mater. Res., Vol. 13, No. 2, Feb 1998