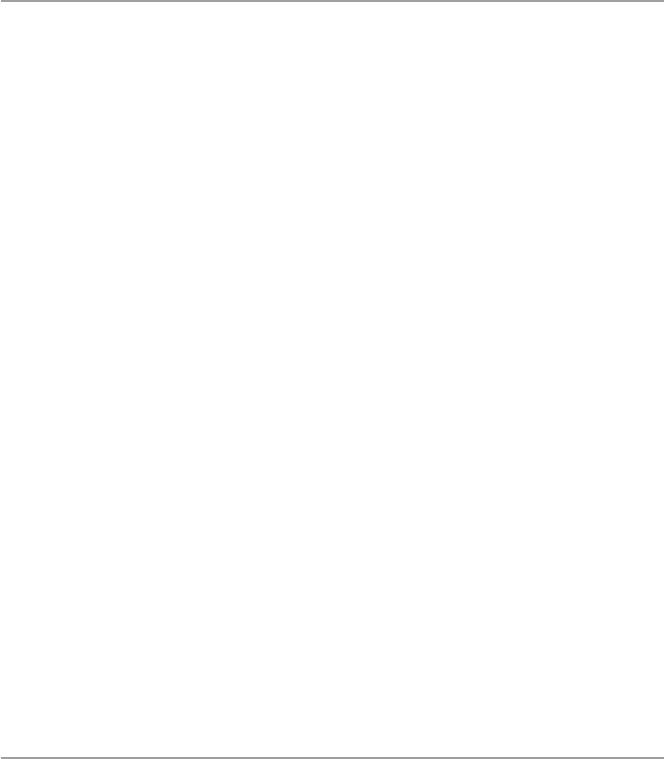
331
The direct assay in Fig.14.5c was established by rst forming an amine-reactive
self-assembly monolayer (SAM) incubating the nanochips in ethanolic solution of
0.4mM of 10-carboxy-1-decanethiol and 1.6mM of 1-octanethiol for 12h at room
temperature, then washed thoroughly with pure ethanol and dried in room tempera-
ture. Then the chip was incubated in a mixture of sulfo-N-hydroxysuccinimide
(sulfo-NHS) and 1-ethyl-3-(3-dimethylaminopropyl) carbodiimide (EDC) (Bio-
Rad, Hercules, CA, USA) to activate the carboxylic group of the SAM for 15min.
Next, 50μL of 200μg/mL anti-troponin antibody 560 (Hytest, Finland) in acetate
buffer at pH4.5 was spotted on the chip surface and incubated for 30min. Finally,
the sensor chip was immersed in 1 M of ethanolamine-HCl solution (Bio-Rad,
Hercules, CA, USA) for 15min to deactivate the unreacted esters, followed by a
rinse with deionized water and dried with nitrogen gas at room temperature. The
sensing chip is blocked by 1% bovine serum albumin (BSA) solution for 15min to
prevent the nonspecic binding, then 50 μL of human cTnI standard in serum
(Phoenix Pharmaceuticals, CA, USA) in the concentrations of 75, 30, 7.5, or 2.5ng/
mL was applied and incubated for 30min. The sensor chip was rinsed with PBS
solution three times after incubation, and inserted into the measurement cell con-
taining PBS buffer to record its transmission spectrum.
The binding of the cTnI on anti-troponin antibody on the sensor chip will shift
the plasmonic peak at the wavelength of 644.07–654.85nm (Fig.14.5d). The peak
at 644.07nm is the α peak in Fig.14.2b which has the plasmonic eld concentrated
on the gold–water interface where the bioassay is anchored. Thus the LSPR bio-
sensing for this plasmonic peak monitoring is relatively sensitive. Figure 14.5e
shows the characterization curve for cTnI detection, with error bars obtained by
measuring the same troponin concentrations with three individual nanochips.
The performance of this point-of-care device is found to have a full half- magnitude
width (FHMW) of 32.84nm, gure of merit (FOM) of 10.5, σ of signal at 0.017nm,
and signal-to-noise ratio equals to 256 (at 7.5ng/mL troponin in serum), and the limit
of detection (LOD) based on three times of σ is identied to be 0.55ng/mL.
14.4 Point-of-Care Device Based onFluorescent Label
Enhancement
We have developed several sandwich bioassays based on plasmonic-enhanced uo-
rescent labels to respectively detect the prostate specic antigen (PSA) for prostate
cancer, thrombin for blood clots, and procalcitonin (PCT) for sepsis. When estab-
lishing such a bioassay, a few factors are considered. The rst is that the distance of
the uorescent label should be around 10–20nm to the gold nanostructure surface,
so that the dyes are enhanced by the plasmons instead of being quenched by the
strong plasmons. The second is that the excitation wavelength of the dye must be
close or slightly shorter than the LSPR generated by the gold nanostructures, so as
to make sure that the light being used to excite the uorescent dyes are amplied by
the plasmon. Here we use quantum dot (QD) as labels, because compared with
organic uorescent dyes, the QDs are about 20 times brighter, and they have a
broadband of excitation wavelength range (Song etal. 2015a).
14 Point-of-Care Device withPlasmonic Gold Nanoarray Sensing Chip